Selected Research Highlights
➤ Adaptive cognition implemented with a context-aware and flexible neuron for next-generation artificial intelligence
P. Jadaun, C. Cui, S. Liu and J. A. Incorvia
Proceedings of the National Academy of Sciences Nexus 1, 1-18 (2022)
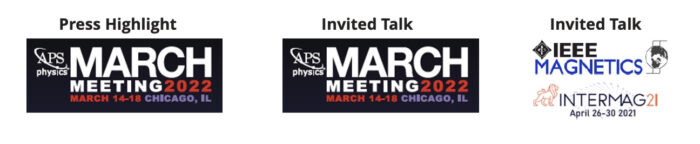
‘Press Highlight’ of APS March Meeting, 2022 (Top 0.3% out of 10,000 technical papers)
Media coverage – Dept. of ECE, UT Austin
Showcased in invited talk – APS March Meeting, 2022
Showcased in invited talk – Intermag, 2021
Bio-inspired neuromorphic computing aims to replicate the sophisticated intelligence abilities of the brain. A remarkable and sought-after ability of the brain is its adaptive intelligence, whereby the brain regulates its functions “on the fly” to respond to complex and ever-changing environments, contexts, goals and rewards. This adaptive intelligence shown by the brain originates in the adaptive nature of the biological neuron. An adaptive neuron is one in which the neuron’s properties, including its state, its transfer function and its state space, can be altered or modulated “on-the-fly”.
The brain’s adaptive abilities are critical for a wide range of high-level intelligence (cognitive) functions. Despite their importance, these adaptive abilities have yet to be realized in neuromorphic hardware. In this work, an adaptive oscillatory neuron is designed using a lattice of magnetic textures called skyrmions.
This work is published in PNAS Nexus and has been selected as ‘Press Highlight’ at the APS March Meeting 2022. It has also been selected for invited talks at APS March 2022 and Intermag 2021.
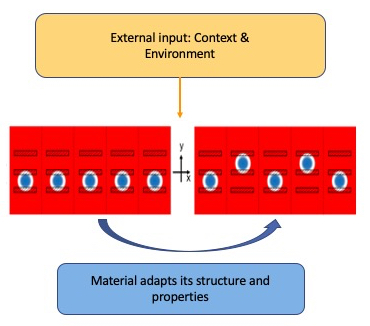
Skyrmions are used as they offer a rich physical space for hardware-aware computing, including making use of skyrmion core oscillations and skyrmion-skyrmion interactions. The behavior of arrays of skyrmions can be manipulated by multiple inputs, such as currents and electromagnetic waves.
A charge current is used to reconfigure the skyrmion lattice, by altering the positions of the skyrmions. This reconfiguration alters the neuron’s internal structure, thereby modulating the neuron’s state, its dynamics, and its transfer function and mimicking the adaptive intelligence shown by a biological neuron.
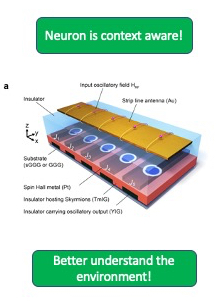
As a result of its adaptive abilities, this neuron demonstrates 3 high-level intelligence functions.
- It is the first hardware-based neuron to understand and respond to underlying context, which allows the neuron to make complex, high-level decisions while considering multiple factors and make predictions from small datasets.
- The neuron is also the first to realize cross frequency coupling (CFC) in hardware, another high-level intelligence ability. CFC can help achieve general artificial intelligence (AI) using energy-efficient and compact artificial neural networks (ANNs) that lead to smart agents on the edge impacting everyday life.
- Furthermore, this neuron is one of the first hardware-based neurons to demonstrate fusion of information called feature binding. Feature binding is a mechanism by which the brain combines or fuses disparate features of an object to form a coherent perception of the entire object and can have significant impact on autonomous vehicles (e.g., camera and radar fusion), neuro-prosthesis, human-machine interaction (e.g., vision and tactile fusion), wearable health technology etc.
To demonstrate its potential, an artificial neural network (ANN) built with this adaptive neuron is used to carry out context-aware diagnosis of breast cancer. The adaptive ANN outperforms state-of-the-art, non-adaptive ANNs by diagnosing cancer with higher accuracy, while learning from smaller amounts of data, and using an energy-efficient and smaller network size. Neuromorphic implementation of these cognitive abilities can have transformative impact on a multitude of fields including biomedicine, human-machine collaboration, advanced manufacturing, edge computing, autonomous vehicles, wearable health technology and climate control.
More generally, hardware-based adaptive neurons such as the one presented in this work are a fundamental advancement over state-of-the-art non-adaptive neurons and can mitigate several critical challenges facing contemporary ANNs. Modern ANNs suffer from a variety of challenges as they demand large volume of training data, unsustainably large energy and chip area when implemented on CMOS circuits and are highly specific or non-general in their applications. In contrast, the adaptive hardware-based neuron proposed in this work can enable adaptive ANNs that learn faster from smaller datasets, have compact architectures, are energy-efficient, are fault-tolerant and can realize general artificial intelligence.
➤ Rational design principles for giant spin Hall effect in 5d-transition metal oxides
P. Jadaun, L. F. Register and S. K. Banerjee
Proceedings of the National Academy of Sciences, 117 (22) 11878-11886 (2020)

UT Research Showcase
Feature Story, Texas Advanced Computing Center (Top 2% out of ~ 3,000 projects conducted)
Media coverage – Dept. of ECE, UT Austin
Spin Hall Effect (SHE) is a mechanism by which a material converts a charge current into a spin current. Unlike competing methods, SHE does not require any external magnetic fields (that are hard to control) or special temperatures to operate. The spin current produced is highly effective at controlling small magnets so as to read, write and store data while using very little energy and area. As a result, SHE is immensely attractive for neuromorphic computing, spin-based quantum computing, and ultra-low power memories. Along with its strong technological importance, SHE is also of fundamental interest owing to its rich physics. However, important questions still remain about the essential factors that determine SHE and technologically useful materials with large values of SHE remain scant.
In this work, I discovered a class of oxide materials (anti-perovskites) that promise SHE values that are 10 times larger than state-of-the-art oxides. Additionally, this work found the five fundamental conditions necessary for large values of SHE in materials. Materials with giant SHE values like those discovered in this work, are critical for realizing SHE-based devices to advance quantum and classical computing. Moreover, the factors uncovered in this work can enhance SHE beyond the values found intrinsically in materials.
As a result, this work was published by PNAS, selected as ‘UT Research Showcase’ and ‘Feature Story’ by Texas Advanced Computing Center. Recently, it has led to the experimental realization of large SHE in oxides with structural distortions that are published in Advanced Materials and Advanced Functional Materials.
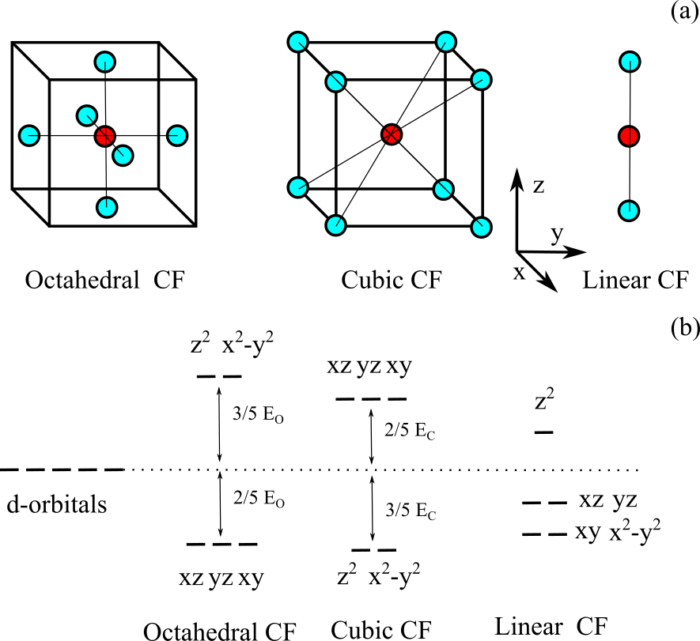
The findings of this work stem, in part, from the key realization that intrinsic SHE is inherently a geometric property that arises from the Berry curvature of the bands in a material. These geometric properties are enhanced, or maximized, when the degrees of freedom (DOFs) of a wave function are freely (maximally) allowed to rotate. Here, we demonstrate that such rotation of the DOFs is maximized under five conditions including:
- Weak crystal fields
- Materials with a weak crystal field, e.g. the linear crystal field (CF) shown in the figure above, have orbital levels that are close in energy which allows the wave functions to rotate enhancing the SHE values.
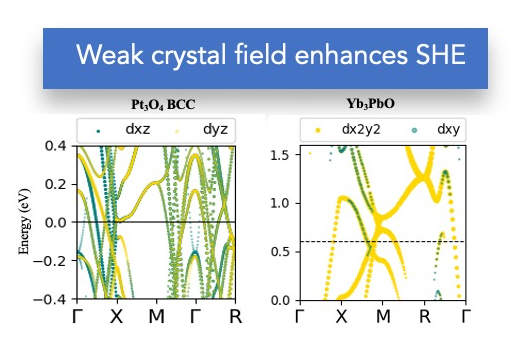
- Structural distortions
- Structural distortions break up energy bands into sub-bands and increase their bandwidth, thereby enhancing rotations between orbitals and consequently SHE values.
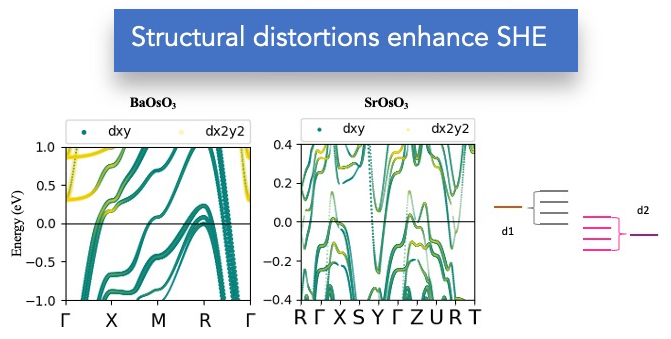
Additionally, we derive three other conditions for enhancing SHE values, namely, optimal positioning of the Fermi level, mixing of Jeff = 1/2 and 3/2 bands, for materials under octahedral or cubic crystal fields with X–Y symmetry, and, in some cases, electron correlations. Together, these conditions represent five rational design principles for the discovery and design of giant SHE. Although derived specifically for SHE in TMOs, our design principles and insights are general enough to apply to transition metal compounds, as well as to other geometric properties like the anomalous Hall effect, orbital Hall effect, valley Hall effect, etc. These design principles acquire enhanced potency due to the unique tunability of transition metal compounds.
Implementing these design principles, this work further presents previously unexplored materials (BCC-Pt3O4 and 5d-antiperovskites) that promise to demonstrate giant efficiencies of conversion of charge current to spin current that are an order of magnitude larger than that reported for any oxide so far. We also enumerate large efficiency values found in a significant number of other oxides. Identifying materials with large conversion efficiency is critical for low-energy spintronic applications, with electrical control of magnetism.
➤ The microscopic origin of DMI in magnetic bilayers and prediction of giant DMI in new bilayers
P. Jadaun, L. F. Register and S. K. Banerjee
Nature Partner Journal Computational Materials 6, 88 (2020)

A skyrmion is a very small magnetic region where the magnetic orientation varies in a swirling pattern about a point. A skyrmion is a compact, topologically protected unit that can be created, moved and annihilated with miniscule currents. As a result, skyrmions are attractive for storing and carrying information in neuromorphic, classical and quantum computing paradigms. Dzyaloshinskii–Moriya interaction (DMI) is often critical to the generation and manipulation of skyrmions. However, there is a fundamental lack of understanding of the origin of DMI or the mechanism by which DMI generates skyrmions in magnetic bilayers. Very little is known of the material parameters that determine the value of DMI. This knowledge is vital for rational design of skyrmion materials and further development of skyrmion technology.
To address this important problem, we investigate DMI in magnetic bilayers using first principles. We present a new theoretical model that explains the microscopic origin of DMI in magnetic bilayers. We demonstrate that DMI depends on two parameters, interfacial hybridization and orbital contributions of the heavy metal. Using these parameters, we explain the trend of DMI observed. We also report four new materials systems with giant DMI and new designs for magnetic multilayers that promise enhanced stability of skyrmions. Our results present a notably new understanding of DMI, uncover highly promising materials and put forth pathways for the controlled generation of skyrmions.
This discovery of skyrmions with enhanced stability can enable real-world devices for neuromorphic and quantum computing. Moreover, understanding the origin of skyrmion stability can engender schemes for localized generation and external manipulation of skyrmions. As a result of its impact, this work was published in Nature Partner Journal Computational Materials and has led to experimental realizations of skyrmions that are stable even in the absence of external magnetic fields.
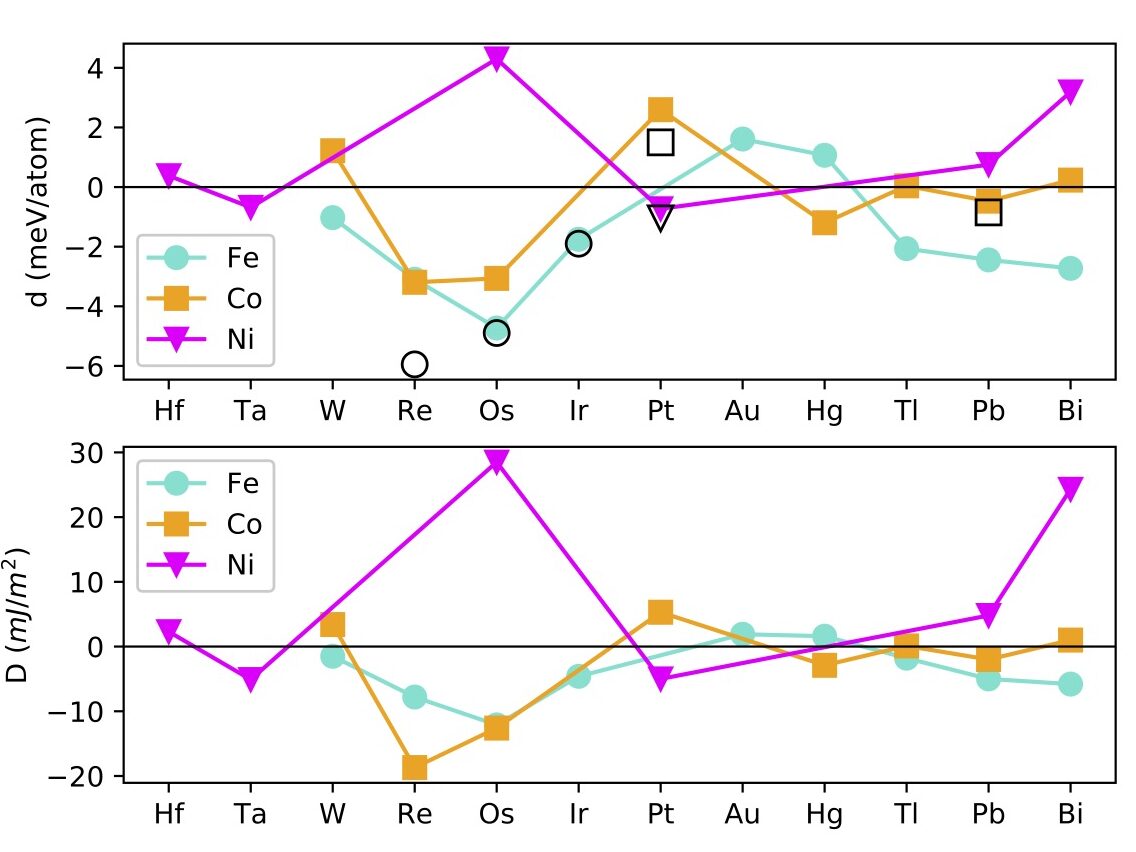
We calculated values of DMI in a range of bilayers of heavy metal (HM)/ferromagnet (FM) and they are plotted in the figure above. A significant result is the giant DMI seen in six bilayers, namely, Re/Fe, Os/Fe, Re/Co, Os/Co, Os/Ni and hexagonal Bismuth(hBi)/Ni. These materials show a DMI (d) up to twice the largest known values at the time of publication, i.e., of 1.5 meV/atom for Co/Pt, −1.9 meV/atom for Ir/Fe and −1.04 meV/atom for Ir/Co.
Further, we showed that the strength of DMI (d) is principally determined by the HM–FM hybridization. To demonstrate this, we examined the projected density of states (p-DOS)and relative band alignments between the HM and FM layers.
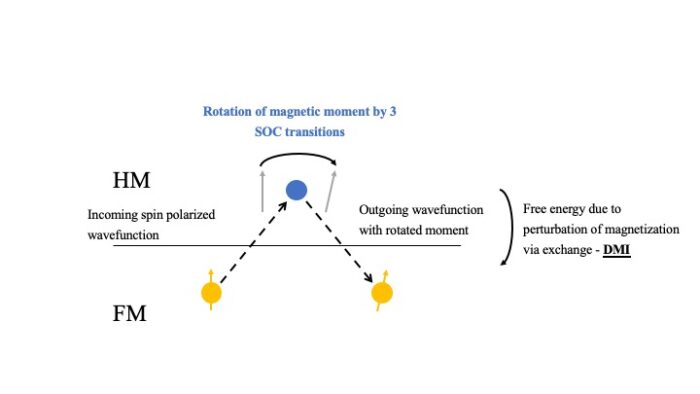
To explain the origin of the sign of DMI, we developed a new model for the mechanism by which spin orbit coupling (SOC) generates magnetic textures, and demonstrated that the sign of DMI is controlled by the relative presence of HM orbitals in the bandstructure.
We first prove that DMI originates in three off-diagonal, spin-mixing terms of the spin orbital coupling (SOC) operator. To explain this model, we consider a wavefunction hopping across the HM/FM interface. In the presence of SOC, whenever the electron hops to the HM atom, the three SOC spin mixing terms cause d-orbital transitions and rotate its magnetic moment (creating a swirling spin texture). As this electron hops to the FM, its rotated magnetic moment perturbs the wavefunctions of the FM via exchange interaction, altering the free energy and generating DMI. The stronger the HM–FM hybridization, the stronger the perturbation of energy due to the hopping electron, the larger the DMI. Together, the sign of DMI is decided by the interplay between these three SOC spin mixing terms and the strength of DMI is decided by the HM–FM hybridization.
➤ Topological Classification of Crystalline Insulators with Point Group Symmetry
P. Jadaun, D. Xiao, Q. Niu, S. K. Banerjee
Phys. Rev. B 88, 085110 (2013)
Polarization is a ubiquitous property and is the corner stone of ferroelectricity. Despite being a fundamental quantity in condensed-matter physics, the theoretical underpinnings of polarization were poorly understood. Here, we solved a fundamental problem of why polarization could only adopt discrete or quantized values, by showing that polarization was a topological property.
First, this discovery helped us better understand the origins of polarization.
Second, it enabled us to classify materials into topological classes. In this work, we completely classified all 2D crystals into their symmetry protected topological classes. Understanding which materials belong to which topological class, and how that affects their properties, is a deeply important problem.
Third, we predicted novel candidates for topological crystalline insulators. These are materials where the topological class of a material is protected by its crystal symmetry. Our theoretical predictions led to the experimental realization of a new topological crystalline insulator found in 2D transition metal dichalcogenide in work that is now published in Nature Partner Journal 2D Materials and Applications.
The topology of a material describes the global, and not local, state of its electrons. While local properties vary easily and continuously, a global property can only be altered by large perturbations, and that too only in discrete steps. Our discovery that polarization is a topological index explained why it was quantized. We also showed that in this case topology was restricted by symmetry, hence, a given symmetry could only allow for certain polarization values.